Purpose
- Learn the answers to frequently asked questions about best practices for ventilation systems.
- Understand more details on complex information on improving ventilation.
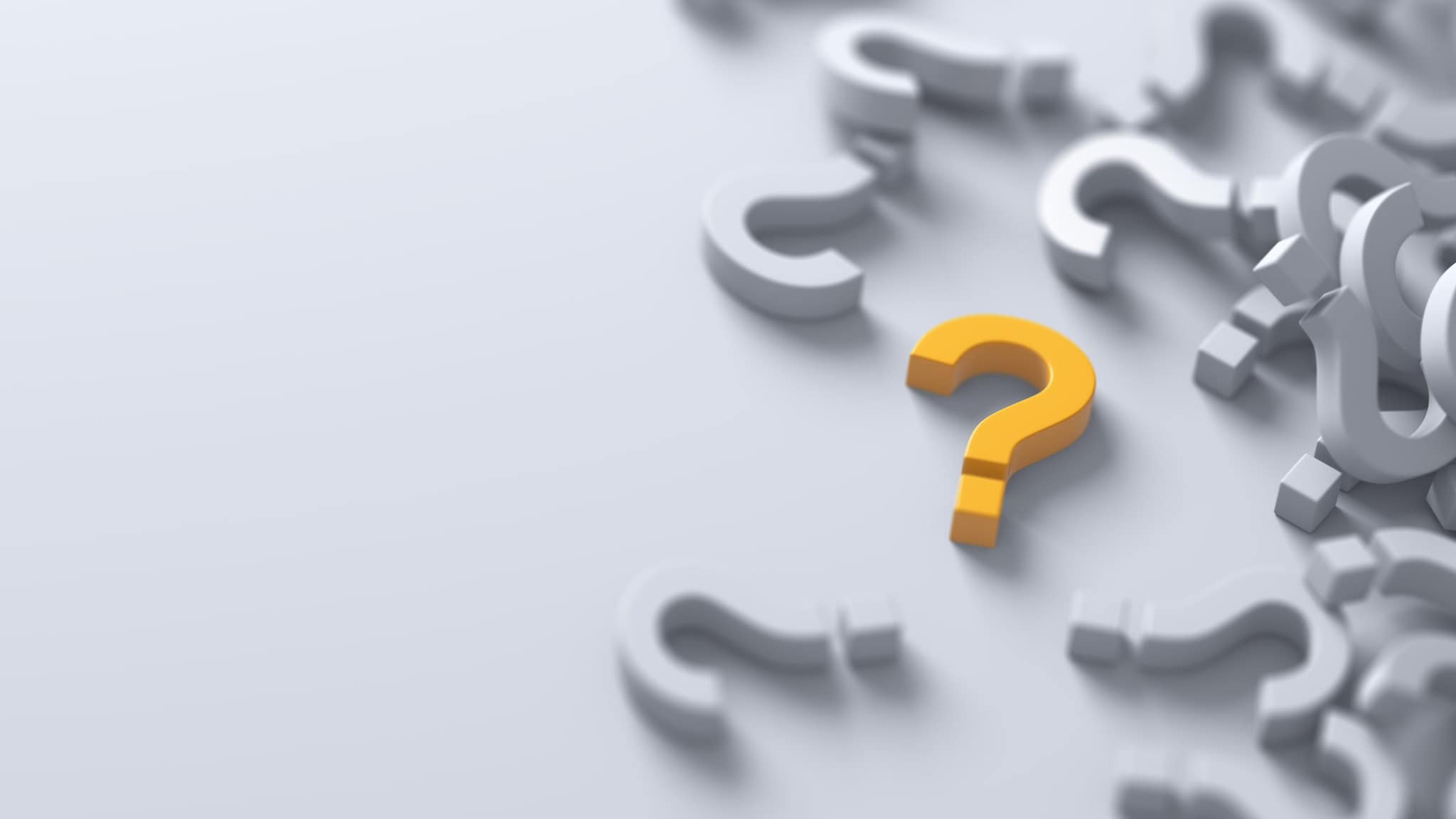
Air flow
Some viral particles might travel from one space to another through a heating, ventilation, and air conditioning (HVAC) system. However, the risk for receiving an infectious dose will depend upon the specific disease as well as any filtration and air cleaning equipment used within the system.
While airflow distribution within an occupied space is an important factor worth evaluation (see FAQ #4), outbreak investigations have often found that respiratory virus transmission occurs between an infected person and uninfected persons in the same space. Viral RNA has sometimes been found on return air grilles, in return air ducts, and on HVAC filters, but detecting viral RNA alone does not imply that the virus was capable of transmitting disease. While airflows within a particular space may help spread disease among people in that space, there is minimal definitive evidence for most diseases that viable virus has been transmitted through an HVAC system to result in disease transmission to people in other spaces served by the same system.
Healthcare facilities have ventilation requirements in place to help prevent and control infectious diseases that are associated with some healthcare environments. For more information, see the CDC Recommendations for Environmental Infection Control in Health-Care Facilities.
Non-healthcare (e.g., businesses and schools) building owners and managers should, at a minimum, maintain building ventilation systems according to state and local building codes and applicable guidelines. Ensuring appropriate outdoor air and ventilation rates is a practical step to ensure good indoor air quality. However, these codes do not address infection prevention in non-healthcare buildings and code minimum ventilation may be insufficient to protect indoor occupants under some circumstances (e.g., high incidence rates, occupants near one another, crowded spaces, etc.).
Directional airflow is a protective ventilation concept where air movement flows in a clean-to-less-clean direction.
This ventilation concept is applied to areas where the "clean" environment requires a higher level of protection and/or where the "less-clean" environment has a higher risk of containing airborne contaminants (activities or occupancy by individuals with a higher risk of being infectious). Examples of "clean" spaces might include healthcare facility triage stations or rooms/corridors adjacent to higher risk activities. Examples of "less-clean" spaces might include spaces that contain known/suspect infectious persons or spaces where a known activity has increased likelihood of generating infectious airborne particles.
The creation of directional airflow can be accomplished within a particular space or between two adjacent spaces. This can be done passively, through intentional placement of supply and exhaust heating, ventilation, and air conditioning (HVAC) grilles, or by the intentional creation of pressure differentials between adjacent spaces through specification of offset exhaust and supply air flow rates. Creation of the directional airflow can also be done actively, through the use of fans exhausting through open windows, strategic placement of ductwork attached to in-room HEPA filtration units, or dedicated exhaust systems (installed or portable) that generate a desired airflow by exhausting air out of windows, doorways, or through temporary ducts. In specific settings, specialized local control ventilation interventions that establish the desired airflow directions can also be used (see the NIOSH Ventilated Headboard).
Directional airflows must be evaluated carefully. Testing of the directional airflow effectiveness can be accomplished using visual tracer techniques that use "smoke tubes" or handheld "fog generators." Other tools, such electronic monitors or visual aids to monitor pressure differences can be used when directional airflow is established between two adjacent spaces. To reduce the potential for directing airflow from infectious towards non-infectious space occupants, it is important that the "clean" and "less-clean" space determinations be established using infection control risk assessment considerations.
Yes, carbon dioxide (CO2) monitoring can provide information on ventilation in a given space, which can be used to enhance protection against respiratory virus transmission. Strategies incorporating CO2 monitors range in cost and complexity. However, greater cost and complexity does not always mean greater protection.
Limited information exists regarding a direct link associating CO2 concentration to a risk of respiratory virus transmission. Changes in CO2 concentrations can indicate a change in room occupancy and be used to adjust the amount of outdoor air delivered. However, CO2 concentrations cannot predict who has a respiratory infection and might be spreading the virus, the amount of airborne viral particles produced by infected people, or whether the HVAC system is effective at diluting and removing viral concentrations near their point of generation. Ventilation based on CO2 measurements cannot recognize the increased risk of transmission when multiple room occupants are infected.
In some well-designed, well-characterized, well-maintained HVAC environments, the use of fixed CO2 monitors can be informative. When used, these monitors are often incorporated into demand-controlled ventilation (DCV) systems that are designed with a primary intent of maximizing energy efficiency through reductions in outdoor air delivery. However, during times of high community transmission, guidance is often to deactivate DCV systems and exceed minimum ventilation whenever possible, in addition to enhanced filtration, and other intervention-focused considerations.
Traditionally, CO2 monitoring systems are expensive, require extensive knowledge to accurately install and set up, and require sophisticated control programs to effectively interact with the building heating, ventilation and air conditioning (HVAC) systems in real time. They were not designed to protect building occupants from disease transmission. Fixed-position CO2 monitors measure CO2 concentration as an indicator of the number of people in the space. As the CO2 concentration increases, the HVAC DCV system increases the amount of outdoor air ventilation in the space to dilute CO2 (and vice versa). The number of CO2 sensors, the placement of those sensors, and their calibration and maintenance are collectively a large and complex issue that must not be overlooked. For example, the CO2 concentration measured by a fixed, wall-mounted monitor may not always represent the actual concentrations in the occupied space. If air currents from the room HVAC, or even make-up air from windows, flows directly over this monitor location, the corresponding concentration measurements will be artificially low. If the room has good air mixing, the measured concentration should approximate the true concentration, but rooms are rarely well mixed, particularly in older buildings with aging ventilation systems (or none at all). Also, if an elevated CO2 concentration results in an air flow increase to one room, that air may be "stolen" from other rooms on the same HVAC system. This may result in elevated CO2 concentrations in those other spaces which the HVAC system is unable to control.
A more modest, cost-efficient, and accurate use of CO2 monitoring is the use of portable instruments combined with HVAC systems that do not have modulating setpoints based on CO2 concentrations. The CO2 meter can be purchased for under $300 and its measurements can be collected/logged near the breathing zones of occupied areas of each room. It is critical to select calibrated CO2 meters whose sensors are reliable and accurate to draw meaningful inferences from measured indoor CO2 concentrations. Under this approach, validate that the HVAC system is operating appropriately and is meeting or exceeding code-minimum outdoor air requirements based on current use and occupancy. Next, measure the resulting CO2 concentrations in rooms under as-used conditions using a handheld portable CO2 meter. These observations will be the CO2 baseline concentrations for each room under the HVAC operating conditions and occupancy levels.
One potential target for the baseline concentrations that is used to represent good ventilation is CO2 readings below 800 parts per million (ppm). It is important to note, however, that a single concentration value may not be an appropriate target for all space types and occupancies for the purposes of assessing the ventilation rate (see ASHRAE Position Document on Indoor Carbon Dioxide). Once a target concentration is identified, compare your baseline concentrations to the target concentration. If a baseline measurement is above the target, reevaluate the context under which the measurement was obtained and if warranted, investigate the ability to increase outdoor air delivery. If unable to get below your target CO2 value, increased reliance on enhanced air filtration (including in-room HEPA air cleaners) may be necessary. Once the baseline concentrations are established, take periodic measurements in each space and compare those to the initial baselines. As long as ventilation airflow is unchanged (outdoor air or total air) and occupancy capacity is not increased, future portable CO2 concentrations that exceed 110% of the baseline concentrations indicate a potential problem that should be investigated.
Yes, but with caution.
While fans alone cannot make up for a lack of outdoor air, fans can be used to increase the effectiveness of open windows, as described in the list of ventilation mitigation strategies. Fans can also be used indoors to improve room air mixing. Improved room air mixing helps distribute supplied clean air and dilute viral particle concentrations throughout the room, which reduces the likelihood of stagnant air pockets where viral concentrations can accumulate. As with all fan use during periods of high respiratory disease transmission, take care to minimize the potential to create air patterns that flow directly across one person onto another:
- Avoid the use of the high-speed settings
- Use ceiling fans at low velocity and potentially in the reverse-flow direction (so that air is pulled up toward the ceiling)
- Direct the fan discharge towards an unoccupied corner and wall spaces or up above the occupied zone.
Fans can also enable clean-to-less-clean directional airflow. Such applications should be evaluated closely to avoid unintended consequences and only adopted when supported by a safety risk assessment.
Barriers can help or barriers can hurt. It depends on where and how they are deployed.
Barriers can physically separate spaces that are next to each other. When used for infection control, the barrier is intended to prevent someone on one side of the barrier from exposing a person on the other side of the barrier to infectious fluids, droplets, and particles. Whether a barrier interferes with improved ventilation depends on how it is installed. Protective barriers can sometimes help improve ventilation, but they can sometimes hinder ventilation too. Sometimes they have no effect on ventilation.
Protective barriers can assist with improved ventilation when used to facilitate directional airflows or desired pressure differentials between clean and less-clean spaces. The barrier can be aligned with the intended airflow to help direct it towards a desired location, such as an HVAC return air grille or an in-room HEPA air cleaner inlet. Example scenarios for this type of barrier deployment include those where there is a likely source of potentially infectious aerosols, such as a dental operatory or urgent care exam room.
Alternatively, the barrier might be placed between two areas to better isolate one side of the barrier from the other. In this configuration, the barrier can also assist the HVAC design scheme in establishing a desired pressure differential between the adjacent spaces. If necessary, small pass-through openings or a retractable panel incorporated into the barrier can allow transfer of physical objects from one side to the other. Examples where this type of barrier application might be applied include a medical intake triage station or a pharmacy pick-up window .
When not carefully installed, barriers can sometimes hinder good ventilation. Barriers can unintentionally interrupt the airflow distribution within a space, thus allowing a concentration build-up of human-generated or other aerosols that may remain suspended in the air for minutes to hours. In this case, people could be exposed to higher concentrations of infectious aerosols than they would without the barriers in place. The larger the barrier, the greater the likelihood that this may occur. To reduce this likelihood, ensure that barriers are correctly positioned for the anticipated occupancy and that they are no larger than necessary to prevent direct transfer of respiratory droplets that could "spray" directly from one person onto another.
Any time barriers are deployed, airflow distribution testing with tracer "smoke" or handheld fog generators should be conducted. This testing can assist in evaluating airflow distribution within the occupied spaces. If stagnant air pockets are seen to occur, barrier redesign or reorientation can help to minimize the occurrence. Airflow distribution modifications such as adjusting the positioning of supply air louvers or the discharge of in-room air cleaners can also assist in eliminating the development of stagnant air pockets.
Note: The installation of barriers should not interfere with emergency egress or with the protective function of fire sprinklers. When in doubt, consultation with local code and fire officials is encouraged.
ASHRAE Standard 241 (S241) was published in July 2023 and is entitled "Control of Infectious Aerosols". No, it does not conflict with CDC's Ventilation Mitigation Strategies; in fact, the two can complement each other.
The S241 is the result of an intense effort by ASHRAE professionals to "establish minimum requirements aimed at reducing the risk of disease transmission through exposure to infectious aerosols in new buildings, existing buildings, and major renovations." A key requirement is the equivalent clean airflow per person for infection risk mitigation (ECAi) to be delivered to the breathing zones of room occupants. The amount of air required varies by room type and is based upon modeled assumptions related to the occupancy and activity within the room. These minimum clean air requirements apply only when the building is operating under Infection Risk Management mode (IRMM). The standard does not specify who decides when IRMM is in effect.
One misperception is that the S241 clean air requirements conflict with the "5 or more ACH" ventilation recommendations from CDC. The S241 is written with a compliance focus. Jurisdictions with legal authority who adopt S241 can then enforce its requirements. In contrast, CDC's Ventilation Mitigation Strategies are voluntary, offering improved ventilation strategies for those seeking increased protection from respiratory aerosol exposures. Both S241 and CDC's Ventilation Mitigation Strategies emphasize the importance of bringing building HVAC systems up to current code requirements. Ventilation improvements then supplement the current code benchmark to provide additional clean air. For buildings or jurisdictions where S241 has been adopted, the Table below identifies when airflow delivery strategies might be based on CDC's "5 or more ACH" guidance vs S241's ECAi minimum requirements:
Condition
Applicable Guidance/Standard (airflow)
Comment
Day-to-day, non-IRMM
CDC
When S241 ECAi < CDC 5+ ACH, S241 ECAi may sometimes be appropriate for day-to-day operation.*
IRMM declared by authority having jurisdiction
S241
When S241 ECAi > CDC 5+ ACH, S241 ECAi air flows will provide more protection.
When S241 ECAi < CDC, S241 ECAi is the minimum ventilation requirement for the IRMM condition. However, the higher CDC 5+ ACH ventilation rate may be voluntarily applied for added protection.
*The CDC guidance includes numerous caveats acknowledging some space type and occupancy variations may not require 5+ ACH for sufficient day-to-day ventilation. One example is large warehouses with few people. In these cases, S241 ECAi flow rates could be viewed as sufficiently protective for day-to-day operation.
Dilution, filtration, and air treatment
The time required for dilution will depend on room ventilation airflow and its effectiveness.
While large droplets (100 micrometers [µm] and larger) will settle to surrounding surfaces within seconds, smaller particles can stay suspended in the air for much longer. It can take several minutes for particles 10 µm in size to settle, while particles 5 µm and smaller may not settle for hours or even days. Dilution ventilation and particle filtration are commonly used to remove these smaller particles from the air. Larger particles can also be removed using these strategies, but since they fall out of the air quickly, they might not have a chance to get captured by filtration systems.
Delivering (or exhausting) an amount of air that is equal to a room's volume is called an "air change." Delivering this amount of air over a 60-minute period is called an "air change per hour" (ACH). The time required to remove airborne particles from a space can be estimated using Table B.1 in the CDC's Recommendations for Environmental Infection Control in Health-Care Facilities (2003). The estimates assume the source of infectious particles is no longer present in the space. The estimates in the table are based upon the rate that particle-free air is delivered to the room and the desired removal efficiency (99% or 99.9%). The particle-free air, measured in air changes per hour (ACH), can be uncontaminated supply air or the clean exhaust from a High Efficiency Particulate Air (HEPA) fan/filtration system [See HEPA filtration discussion below].
Although there are some highly contagious airborne diseases (like measles) where CDC provides specific guidance for 99.9% clearance wait times, the general recommendation in CDC's Recommendations for Environmental Infection Control in Health-Care Facilities is to wait to allow for a 99% reduction of any generated airborne particles before re-entering the room.
In the absence of guidance specifying a longer wait period for a particular respiratory disease , the wait time associated with 99% clearance is an appropriate target for healthcare and other spaces where an infectious occupant is reasonably anticipated to be present. Regardless of whether the 99% or 99.9% column on Table B.1 is used, the value in the table is usually an under-estimation of the actual dilution clearance time as noted in the table's footnotes which include the following statement: "The times given assume perfect mixing of the air within the space (i.e., mixing factor = 1). However, perfect mixing usually does not occur. Removal times will be longer in rooms or areas with imperfect mixing or air stagnation." Appropriate use of Table B.1 to establish clearance times from any space requires multiplying the time in the table by a mixing factor (k) that ranges between 1 and 10. This factor represents how well the ventilation system mixes and dilutes the concentration of airborne particles within the room.
As a rule of thumb, rooms with higher airflow rates (6 ACH and higher) and good placement of supply and exhaust grilles (hospital airborne infection isolation rooms) are considered to have "good" mixing and thus a mixing factor of k = 3 is often used for these spaces. In that case, the time identified from Table B.1 should be multiplied by 3 to determine the actual clearance time prior to re-entry. Nonventilated or poorly ventilated spaces have typical values of k ranging from 8 to 10. Increased ACH generally leads to reductions in k, although k can also be reduced by the use of a fan in the space, which does not have an impact on ACH. Ultimately, wait times can be reduced by increasing ACH, reducing k, or a combination of both.
Example 1. Given: A room measuring 12 feet x 10 feet with a ceiling height of 10 feet is served with a 100% outdoor air ventilation system that delivers 65 cubic feet per minute (cfm) of supply air (Qs = 65 cfm) and exhausts 80 cfm of air from the room (Qe = 80 cfm). The room has average air mixing, so assign k = 5.
Question: How much time is required to reduce the airborne concentration by 99 percent?
Solution: Since Qe is larger than Qs by 15 cfm, the heating, ventilation, and air conditioning (HVAC) system is pulling 15 cfm of air into the room from adjacent areas (i.e., the room is under negative pressure). For this example, the 15 cfm of transfer air is assumed to be free of infectious airborne particles. The clean volumetric air flow rate (Q) is the larger value between Qs and Qe, so Q = 80 cfm. Calculate the air changes per hour:
ACH = [Q x 60] / (room volume) = (80 cfm x 60) / (12' x 10' x 10') = 4800/1200 = 4.0 ACH
Using Table B.1 the perfect mixing wait time based on 4 ACH and a 99% reduction of airborne particles is 69 minutes.
Using the mixing factor of 5, the estimated wait time for 99% reduction of airborne contaminants in the room is 5 x 69 = 345 minutes or 5 hours and 45 minutes.
Note: Determining the true value of the mixing factor is difficult and requires special equipment to measure air flows and conduct tracer gas decay testing. Thus, conservative estimates of k are often used (as described above). Also, the addition of an air cleaning device (e.g., an in-room HEPA filtration unit) within the same room will reduce the wait time. The flow rate from the air cleaning device can be added to Q determined above, which will increase the overall ACH in the room. The air movement created by the air cleaning device can also decrease the value of k. Together, the increased ACH and decreased k can help substantially reduce wait times. See Example 2 at the bottom of FAQ #5 for more information, including an example of the calculations.
Yes, filters with higher collection efficiencies can provide significant reductions in viral particle concentrations.
Filters for use in heating, ventilation, and air conditioning (HVAC) systems are generally tested under procedures outlined in ANSI/ASHRAE Standard 52.2-2017-Method of Testing General Ventilation Air-Cleaning Devices for Removal Efficiency by Particle Size. This standard was developed by ASHRAE, a global society focused on building systems, indoor air quality, and sustainability in the built environment. Based on the filtration efficiency determined by the testing procedures, filters are assigned a Minimum Efficiency Reporting Value (MERV). The MERV provides a measure of the "filter efficiency" over the range of particle sizes prescribed in the test procedure. MERV values range from 1 to 16 and higher MERV values correspond to more efficient filters.
Research shows that viral particles associated with respiratory disease are extremely small, predominantly measuring under 1 micrometer (µm) in their longest dimension. However, the virus generally does not travel through the air by itself. These viral particles are human generated, so the virus is trapped in respiratory droplets and droplet nuclei (dried respiratory droplets) that are larger than an individual virus. Most of the respiratory droplets and particles exhaled during talking, singing, breathing, and coughing are less than 5 µm in size. CDC recommends using MERV 13 filters or filters with the highest efficiency possible, without having detrimental effects on overall HVAC system performance. ASHRAE has similar guidance, which is to "use combinations of filters and air cleaners that achieve MERV 13 or better levels of performance for air recirculated by HVAC systems." The simplest way to meet this guidance is to use a MERV 13 filter in your appropriately-designed HVAC system, if the system is able to operate properly with it installed. Alternatively, other effective air cleaning technologies can also be used to help meet this performance target. A MERV 13 filter is at least 50% efficient at capturing particles in the 0.3 µm to 1.0 µm size range and 85% efficient at capturing particles in the 1 µm to 3 µm size range. Collectively these particles are capable of remaining airborne for hours and are most associated with deep lung penetration. A MERV 14 filter is at least 75% and 90% efficient, respectively, at capturing those same particles. Efficiencies for MERV 15 and MERV 16 filters are even higher. Thus, the recommended filters are significantly more efficient at capturing particles of concern than a commonly-used MERV 8 filter, which is only around 20% efficient in the 1 µm to 3 µm size range and is not rated for capture efficiency of the smaller 0.3 µm to 1.0 µm particles.
Increasing filtration efficiency can increase the pressure drop (resistance to air flow) across the filters. This can lead to increased fan energy, reduced airflow rates, and/or issues controlling indoor temperature and relative humidity levels. Scientific developments in filter design and manufacturing have reduced the amount of the increased pressure drop and its resulting impact on HVAC operations, but not all filters have adopted the newer technology. Prior to a filtration upgrade, the specific filters under consideration should be investigated for their pressure drop ratings at the flow rate(s) of intended use and the potential impacts of that pressure drop evaluated against the capabilities of the existing HVAC system.
High-efficiency particulate air (HEPA) filters are even more efficient at filtering human-generated infectious particles than MERV 16 filters. However, outside of a few unique applications, HEPA filters are rarely used in central HVAC systems. [See FAQ #5 on HEPA filters and in-room HEPA air cleaners to learn more about them and their application in protective air cleaning].
By definition, a High Efficiency Particulate Air (HEPA) filter is at least 99.97% efficient at capturing particles 0.3 µm in size. This 0.3 µm particle approximates the most penetrating particle size (MPPS) through the filter. HEPA filters are even more efficient at capturing particles larger and smaller than the MPPS. Thus, HEPA filters are no less than 99.97% efficient at capturing human-generated viral particles.
Research shows that the particle size of respiratory viruses is very small. For example, SARS-CoV-2 is around 0.1 micrometer (µm). However, respiratory viruses generally do not travel through the air by themselves. These viral particles are human-generated, so the virus is trapped in respiratory droplets and droplet nuclei (dried respiratory droplets) that are larger. Most of the respiratory droplets and particles exhaled during talking, singing, breathing, and coughing are less than 5 µm in size.
The excellent capture efficiency of HEPA filters comes at a cost, namely the significant pressure drop (and energy) required to move air through the HEPA filter. For this reason, most traditional HVAC systems are not able to use HEPA filters and are limited to the use of less-efficient filters. To account for the increased pressure requirements, HEPA filtration units often combine a HEPA filter with a dedicated fan system.
In-room HEPA air cleaners that combine a HEPA filter with a powered fan system are a preferred option for auxiliary air cleaning, especially in higher risk settings such as health clinics, vaccination, and medical testing locations, workout rooms, or public waiting areas. Other settings that could benefit from in-room HEPA filtration can be identified using typical risk assessment parameters, such as community incidence rates, facemask compliance expectations, occupant vulnerability and room occupant density. While these systems do not bring in outdoor dilution air, they are effective at cleaning air within spaces to reduce the concentration of airborne particulates, including particles associated with a respiratory virus. Thus, they give equivalent air exchanges without the need for conditioning outdoor air.
In choosing an in-room HEPA air cleaner, select a system that is appropriately sized for the area in which it will be installed. This determination is made based on the air flow through the unit, which is typically reported in cubic feet per minute (cfm). Many portable HEPA filtration units are assigned a Clean Air Delivery Rate (CADR) (See EPA's Guide To Air Cleaners In The Home), which is noted on a label in the operator's manual, on the shipping box, and/or on the filtration unit itself. The CADR is an established standard defined by the Association of Home Appliance Manufacturers (AHAM). Participating portable air cleaner manufacturers have their products certified by an independent laboratory, so the end user can be assured it performs according to the manufacturer's claims. The CADR is generally reported in cfm for products sold in the United States. The paragraphs below describe how to select an appropriate air cleaner based on the size of the room in which it will be used. The procedure below should be followed whenever possible. If an air cleaner with the appropriate CADR number or higher is not available, select the unit with the highest available CADR rating. The unit will still provide incrementally more air cleaning than having no air cleaner at all.
In a given room, the larger the CADR, the faster it will clean the room air. Three CADR numbers are given on the AHAM label, one each for smoke, dust, and pollen. The smoke particles are the smallest, so that CADR number applies best to viral particles related to respiratory diseases. The label also shows the largest room size (in square feet [ft2]) that the unit is appropriate for, assuming a standard ceiling height of up to 8 feet. If the ceiling height is taller, multiply the room size (ft2) by the ratio of the actual ceiling height (ft) divided by 8. For example, a 300 ft2 room with an 11-foot ceiling will require a portable air cleaner labeled for a room size of at least 415 ft2 (300 × [11/8] = 415).
The CADR program is designed to rate the performance of smaller room air cleaners typical for use in homes and offices. For larger air cleaners, and for smaller air cleaners whose manufacturers choose not to participate in the AHAM CADR program, select a HEPA unit based on the suggested room size (ft2) or the reported air flow rate (cfm) provided by the manufacturer. Consumers might take into consideration that these values often reflect ideal conditions which overestimate actual performance.
For air cleaners that provide a suggested room size, the adjustment for rooms taller than 8 feet is the same as presented above. For units that only provide an air flow rate, follow the "2/3 rule" to approximate a suggested room size. To apply this rule for a room up to 8 feet tall, choose an air cleaner with an air flow rate value (cfm) that is at least 2/3 of the floor area (ft2). For example, a standard 300 ft2 room requires an air cleaner that provides at least 200 cfm of air flow (300 × [2/3] = 200). If the ceiling height is taller, do the same calculation and then multiply the result by the ratio of the actual ceiling height (ft) divided by 8. For example, the 300 ft2 room described above, but with an 11-foot ceiling, requires an air cleaner that can provide at least 275 cfm of air flow (200 × [11/8] = 275).
While smaller HEPA fan systems tend to be stand-alone units, many larger units allow flexible ductwork to be attached to the air inlet and/or outlet (note that larger ducted units don't fall under the "room air cleaner" description and may not have a CADR rating). Using ductwork and placing the HEPA system strategically in the space can help provide desired clean-to-less-clean airflow patterns where needed. Ducted HEPA systems can also be used to establish direct source capture interventions for patient treatment and /or testing scenarios (See CDC/NIOSH discussion on Ventilated Headboard). Depending on the size of the HEPA fan/filter units and how the facility in which they are being used is configured, multiple small in-room HEPA units deployed to high-risk areas may be more useful than one large HEPA unit serving a combined space.
Example 2. Given: The room described in Example 1 at the bottom of FAQ #2 is now augmented with a portable HEPA air cleaning device with a smoke CADR of 120 cfm (Qhepa = 120 cfm). The added air movement within the room improves overall mixing, so assign k = 3.
Question: How much time is saved to achieve the same 99% reduction in airborne contaminants by adding the portable HEPA device to the room?
Solution: The addition of the HEPA filter device provides additional clean air to the room. Here, the clean volumetric air flow rate (Q) is: Q = Qe + Qhepa = 80 cfm + 120 cfm = 200 cfm.
ACH = [Q x 60] / (room volume) = (200 cfm x 60) / (12' x 10' x 10') = 12,000/1,200 = 10 ACH.
Using Table B.1, the perfect mixing wait time based on 10 ACH and a 99% reduction of airborne particles is 28 minutes.
Using the mixing factor of 3, the estimated wait time for 99% reduction of airborne contaminants in the room is 3 x 28 = 84 minutes. Thus, the increased ACH and lower k value associated with the portable HEPA filtration unit reduced the wait time from the original 5 hours and 45 minutes to only 1 hour and 24 minutes, saving a total of 4 hours and 21 minutes before the room could be safely reoccupied.
Adding the portable HEPA unit increased the equivalent ventilation rate and improved room air mixing. This resulted in over a 75% reduction in time for the room to be cleared of potentially-infectious airborne particles.
Yes, when an appropriate dose of GUV is applied.
Germicidal ultraviolet (GUV), otherwise known as ultraviolet germicidal irradiation (UVGI), is an air and surface treatment tool used in many different settings, such as residential, commercial, educational, and healthcare settings. The technology uses ultraviolet (UV) energy to inactivate (kill) microorganisms, including viruses, when designed and installed correctly.
GUV can inactivate viruses in the air and on surfaces.* The design and sizing of effective GUV treatment systems requires specific knowledge and experience. Seek consultation with a reputable GUV manufacturer or an experienced GUV system designer prior to installing GUV systems. These professionals can assist by properly designing, installing, and commissioning the system for your specific setting.
*Note: While GUV is often an effective supplementary tool for surface disinfection, CDC's recommendation for primary surface disinfection in occupied environments is to follow the CDC/EPA guidance for surface disinfection.
GUV devices, otherwise known as UVGI devices, can take many shapes and sizes. They can also be mounted in various places.
Depending on the manufacturers' claims, these devices may be regulated as pesticide devices by the EPA and require efficacy data. In addition, the site where the product is manufactured may also require registration. See the EPA's Pesticide Devices: A Guide for Consumers for additional information.
Upper-room GUV
Upper-room (or upper-air) GUV uses specially designed GUV fixtures mounted on walls or ceilings to create a treatment zone of ultraviolet (UV) energy that is focused up and away from people. These fixtures treat air as it circulates from mechanical ventilation, ceiling fans, or natural air movement. The advantage of upper-room GUV is that it treats the air closer to and above people who are in the room. Since the 1980s, GUV systems have been widely used for control of tuberculosis (TB). The CDC guidance Environmental Control for Tuberculosis: Basic Upper-Room Ultraviolet Germicidal Irradiation Guidelines for Healthcare Settings provides information on appropriate GUV system design, related safe operation, and maintenance. Based on data from researching multiple human viruses, a UVGI system designed to protect against the spread of TB should be effective at inactivating viral particles associated with a human respiratory disease and therefore prevent spread. While small spaces may require a single GUV fixture, most GUV systems usually require multiple UV fixtures to be effective. For example, a rectangular-shaped waiting room with 10–30 occupants will require 2–3 upper-air GUV fixtures. As part of system installation, care must be taken to control the amount of UV energy directed or reflected into the lower occupied space. Reputable GUV manufacturers or experienced GUV system designers will take the necessary measurements and make any required adjustments to prevent harmful UV exposures to people in the space.
Potential Application: Can be used as an additional layer of protection to treat the air (kill viral particles) in indoor spaces; most useful in spaces that host large gatherings or where the risk of disease transmission is high.
In-duct GUV
In-duct GUV systems are installed within a heating, ventilation, and air conditioning (HVAC) system. These systems are designed to serve one of two purposes:
1) Coil treatment GUV keeps HVAC coils, drain pans, and wetted surfaces free of microbial growth. These devices produce relatively low levels of UV energy. This energy is continually delivered 24 hours a day, which is why they are effective. Coil treatment GUV devices are not designed for treating the air and should not be installed for this purpose.
Potential Application: Can be used to reduce HVAC maintenance and improve operational efficiency within large, commercial HVAC systems or residential HVAC systems; not recommended for inactivating airborne pathogens.
2) Air treatment GUV systems can be effective at applying intense UV energy to inactivate airborne pathogens as they flow within the HVAC duct. HVAC air treatment GUV systems generally require more powerful UV lamps or a greater number of lamps, or both, to provide the necessary GUV required to inactivate pathogens in a short period of time. Air treatment systems are often placed immediately downstream of the HVAC coils. This location keeps the coil, drain pan, and wetted surfaces free of microbial growth and also treats the moving air.
Potential Application: Can be used inside any HVAC system to reduce the concentration of infectious airborne pathogens.
Whole-room GUV
Whole-room GUV (commonly referred to as Far-UV) uses specially designed GUV fixtures mounted on walls or ceilings to create a treatment zone of ultraviolet (UV) energy that extends throughout an occupied space. While standard GUV fixtures emit UV energy at a wavelength around 254 nanometers (nm), far-UV devices use different lamps to emit UV energy at a wavelength around 222 nm. Aside from the wavelength, a major difference between the two technologies is that standard GUV systems are generally designed to avoid exposing people to the UV energy, while many far-UV devices are marketed as safe for exposing people and their direct environment to UV energy. Recent research has indicated 222 nm energy is much safer for humans than once thought. In fact, the American Conference of Governmental Industrial Hygienists (ACGIH) recently increased their Threshold Limit Values (TLVs) 7-fold for eyes and over 20-fold for skin exposed to 222 nm energy. This increase was in response to data showing 222 nm energy does not penetrate the tear layer of the eye or the layer of dead skin (stratum corneum) that protect living skin beneath. Research studies also indicate that far-UV wavelengths can effectively inactivate microorganisms, including human coronaviruses, when appropriate UV doses are applied under experimental conditions. However, there are still some questions about how effective 222 nm energy can be in real occupied spaces against human-generated pathogens when UV exposures are controlled to safe limits.
Far-UV is a promising technology that may well prove to be effective at treating air and surfaces, without some of the safety precautions required for standard GUV. Due to the potential promise this technology represents, there are substantial private and public research activities underway to further validate claims of safety and efficacy. In the near term, whole-room GUV is best viewed as new and emerging technology. Consumers considering an emerging technology such as Far-UV should read FAQ #12 on emerging technologies below.
Potential Application: Air and surface treatment in occupied indoor environments.
CDC recommends using technologies that are known to work and will not cause harm. Be cautious when considering an emerging new technology. Do your homework, to include requesting proof of performance and safety under real-world, as-used conditions.
Depending on the manufacturers' claims, these devices may be regulated as pesticide devices by the EPA and require efficacy data. In addition, the site where the product is manufactured may also require registration. See the EPA's Pesticide Devices: A Guide for Consumers for additional information.
CDC does not provide recommendations for, or against, any manufacturer or product. There are numerous technologies being heavily marketed to provide air treatment to protect against respiratory disease. Common among these are ionization, dry hydrogen peroxide, and chemical fogging. Some products on the market include combinations of these technologies. These products generate ions, reactive oxidative species (ROS, which are marketed using many names), or chemicals into the air as part of the air treatment process. People in spaces treated by these products are also exposed to these ions, ROS, or chemicals. Some research has found these exposures may be harmful under certain conditions, including high concentrations or vulnerable populations.
While variations of these technologies have been around for decades, relative to other air cleaning or treatment methods, they have a less-documented track record when it comes to treating large and fast volumes of moving air within heating, ventilation, and air conditioning (HVAC) systems or even inside individual rooms. This does not necessarily imply the technologies do not work as advertised. However, in the absence of an established body of peer-reviewed evidence showing proven efficacy and safety under as-used conditions, the technologies are still considered by many to be "emerging."
As with all emerging technologies, consumers are encouraged to exercise caution and to do their homework. A manufacturer's reference to regulation and/or product registration, with national or local authorities, does not always imply specific product efficacy or safety. Consumers should research the technology, attempting to match any specific claims against the intended use of the product. Consumers should request testing data that quantitively demonstrates a clear protective benefit and occupant safety under conditions consistent with the intended use. When considering air treatment technologies that potentially or intentionally expose building occupants, the safety data should be applicable to all occupants, including those with health conditions that could be aggravated by the air treatment. In transient spaces, where average exposures to the public may be temporary, it is important to also consider occupational exposures for workers that must spend prolonged periods in the space.
Preferably, the documented performance data under as-used conditions should be available from multiple sources, some of which should be independent, third-party sources. Unsubstantiated claims of performance or limited case studies with only one device in one room and no reference controls should be questioned. At a minimum, when considering the acquisition and use of products with technology that may generate ozone (a gas with potentially harmful health effects), verify that the equipment meets UL 2998 standard certification (Environmental Claim Validation Procedure (ECVP) for Zero Ozone Emissions from Air Cleaners) which is intended to validate that no ozone is produced.
Generally, no. Temperature and humidity can both influence the transmission of infectious diseases, including respiratory viral diseases, but that influence has practical limitations in occupied spaces of buildings.
For example, research on the impact of temperature has shown that SARS-CoV-2, the virus that causes COVID-19, is sensitive to elevated temperatures, with over 99.99% inactivation in only a few minutes at 70°C (158°F). However, this temperature is far outside the limits of human comfort and could damage some building materials. While temperatures lower than 70°C (158°F) were also shown effective, the required exposure time for inactivation increases as the temperature decreases. So, elevated temperatures offer the potential for decontamination of respiratory virus in the air or on surfaces, but the use of increased temperature solely for decontamination is not generally recommended and is not realistic for occupied spaces.
Based on current evidence, it is not clear whether, in practice, increasing humidity could significantly reduce transmission of a respiratory virus disease beyond the reductions that result from using good ventilation and filtration. Several scientific research studies have concluded that respiratory viruses such as influenza and SARS-CoV-2 do not survive as well in environments with higher humidity compared with lower humidity. However, the reasons for this are unclear, and artificial experimental factors like the size of the liquid droplets used in the experiments and the composition of the liquid containing the viruses affect the results. Thus, there is still debate within the scientific community over how much humidity affects virus survival outside of the laboratory. Adding humidity to indoor environments, especially in very cold climates, can also introduce additional challenges to the building environment. CDC and standards-setting organizations like ASHRAE do not make recommendations about controlling indoor humidity to reduce virus survival, although humidity recommendations are made for other reasons, such as prevention of dust mite and mold growth or reduction of static electricity. While not affecting transmission, there are peer-reviewed studies that suggest preventing excessive dryness in the air could help maintain the effectiveness of the human body's immune system.
Yes, when built and used correctly, they can be a protective temporary intervention.
Adding filtration and air movement to a space is generally better than doing nothing when it comes to reducing potential risks from viral particles in the air. Well-constructed do-it-yourself (DIY) air cleaners can serve this purpose. When constructed with great attention to detail, DIY air cleaners have been shown to be effective. Their effectiveness and safety have been supported by the U.S. Environmental Protection Agency (EPA) to reduce wildfire smoke indoors. The particle sizes associated with wildfire smoke include the 1 – 3 micrometer (µm) particles associated with human-generated viral particles, like those that cause respiratory disease. Thus, DIY air cleaners can help reduce exposure to those airborne viral particles.
DIY air cleaners are appropriate during emergencies, for short-term use, or when obtaining commercially-available air cleaners, for whatever reason, is not possible. However, DIY air cleaners should not be used as a permanent, long-term solution for in-room air cleaning. Commercially-available portable air cleaners with high-efficiency particulate air (HEPA) filters are preferred and should be used whenever possible. These units have an established Clean Air Delivery Rate (CADR), which is an established standard defined by the Association of Home Appliance Manufacturers (AHAM). For more information, see the Environmental Protection Agency (EPA) Guide To Air Cleaners In The Home). Participating portable air cleaning manufacturers have their products certified by independent laboratories to assure they perform according to the manufacturer's claims.
DIY air cleaners are generally assembled from box fans and square heating, ventilation and air conditioning (HVAC) filters. Different configurations can be found online that use anywhere from 1 to 5 HVAC filters. A common version, using 4 HVAC filters is often referred to as a Corsi-Rosenthal Box. Regardless of the design, the HVAC filters are sealed to the inlet side of the box fan. Using multiple filters can reduce the resistance to air flow, allowing the fan to move more air when more filters are used in the design. Air cleaning is achieved when the fan pulls contaminated air through the filter and releases the filtered air back into the space through the fan outlet. For these to provide effective air cleaning, they must be assembled properly to eliminate air leaks between individual filters (when multiple filters are used) and where the filters are sealed to the box fan itself. Adequate amounts of duct tape should be used to prevent leaks during assembly. Air leaks reduce the effectiveness of the air cleaner, and this effect can be magnified as the HVAC filter(s) load with dust and the resistance through the filter(s) increases.
In addition to air leaks resulting in filter bypass, the overall performance of DIY air cleaners will depend on the individual box fan and HVAC filters selected. Individual units may perform better or worse than expected. Unless the completed DIY air cleaner is thoroughly tested, a rating akin to the CADR will be unknown. However, the performance of well-assembled DIY units can be approximated by measuring the air exhausted from the fan (using an air flow hood or other similar equipment to measure air flow) and multiplying by the efficiency of the filters at capturing 1 to 3 micrometer (µm) particles. As an example, MERV 13 filters are at least 85% efficient at capturing particles in that size range. A DIY air cleaner capable of moving 300 cubic feet per minute (cfm) of air through MERV 13 filters, would provide 255 cfm (300 cfm × 0.85 = 255 cfm) of "clean" air. However, keep in mind that is simply an approximation and likely represents the best-case performance.
While similar to commercially available HEPA air cleaners, the introduction of DIY air cleaners in a space can introduce new issues that need to be considered. Electrical cords for the fan need to be secured to prevent causing a tripping hazard. The DIY air cleaners can create enough noise to create difficulties communicating verbally. This often causes the units to be turned off or operated at a lower and less-effective fan setting, which defeats the purpose of having the air cleaner in the first place. As with all air cleaners, the DIY units need to be positioned in the room to prevent strong air flows from one person directly over another.